Peter Bruggeman, a professor of Mechanical Engineering at the University of Minnesota, discusses the impact of recent advances in low-temperature plasmas.
Low-temperature plasmas (LTPs) are ionised gases with characteristic electron energies in the eV range (equivalent temperature of 11,600 K) and electron densities that typically remain several orders of magnitude lower than the neutral species. These conditions enable the energetic electrons to generate an abundant amount of reactive species while maintaining near-ambient gas temperatures.
‘Low-temperature plasma’ typically refers to plasmas for which the gas temperature is much less than the electron temperature in contrast with, for example, plasmas in fusion devices or as found in stars that can reach millions of Kelvins. Nonetheless, some LTPs can have gas temperatures that exceed typical combustion temperatures, such as plasmas used for welding.
The chemical reactivity obtained at low gas temperatures is one of the main characteristics of LTPs and enables an exceptionally large variety of applications with an enormous impact on society. LTP is the enabling technology behind the entire microelectronics industry, which allows the processing of materials with up to nanometre resolution for the fabrication of integrated circuits that can be found in computers and other electronic devices. Propulsion systems to control the orbit of satellites surrounding Earth that enable our global communication and navigation (GPS) network also rely on LTPs.
While these two examples refer to LTPs operating at highly reduced gas pressures or even in a vacuum, in the last two decades, LTPs operating in gases at atmospheric pressure have become a significant research focus. The advances in this area extend and build upon broadly used corona treatment technologies for surface functionalisation of materials to enhance adhesion properties and ozone generation for water treatment.
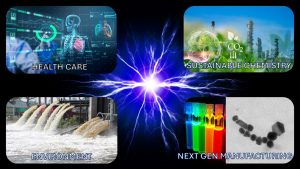
A recent emerging paradigm shift is the vision of electricity-driven LTP as an enabler of chemical transformations addressing a broad range of societal needs.¹ LTPs in this vision are a platform to leverage the increasingly available renewable energy sources and are also compatible with small-scale process implementations. The latter could be a key asset in a future circular economy in which feedstock and products are produced at the point of use.
Major advances in LTP led to a broad range of new developments that continue to broaden the impact of plasmas beyond chemical conversion, including environmental stewardship, energy, sustainability, biotechnology, and medicine.² The rationale and added value that plasmas can bring to these new exciting impact areas are highlighted below (Fig. 1).
Healthcare
In the last two decades, advances in LTPs have led to plasma sources operating at ambient temperatures and pressures that are a copious source of reactive oxygen and nitrogen species (RONS). These plasma sources have shown the ability to combat (antimicrobial resistant) bacteria and viruses, stimulate (chronic) wound healing, and show potential as a novel approach to cancer treatment.³ Animal studies have provided clear evidence that plasma treatments can, in addition to the inactivation of pathogens, also produce an immunogenic response, and in vitro studies have shown that LTPs have the ability to enhance the resistance of cells to viral infection.
The scientific rationale supporting the above emerging findings is centred around reduction-oxidation (redox) reactions that play a major role in many processes during the cell life cycle, dominate the immune system, and are the metabolic driving force for aerobic biology. Plasma-produced RONS are largely the same species as RONS produced in living organisms. Hence, plasma-induced redox chemistry in cells has the potential to regulate all these processes and, dependent on the amount and identity of the delivered RONS, induce different effects on biological systems.
The potential of LTPs to significantly contribute to the mitigation of antimicrobial resistance, food-borne illnesses, and the enormous impact of cancer on our society is a strong motivator for continued research. The goal is to unlock the full potential of LTPs in healthcare far beyond the plasma-based argon-coagulators and surgical devices currently used in hospitals.
Sustainable chemistry
The chemical industry transforms molecules to make fuels, fertilisers, polymers, and many other commodity chemicals. This often involves the dissociation of strong chemical bonds such as those present in CH4 and N2. Incumbent chemical conversion processes leverage thermal catalytic processes at highly elevated temperatures and pressures to achieve that goal.
The required heating for these processes is typically achieved through the combustion of fossil fuels. LTPs have the potential to play a transformative role in chemical conversion as the energetic electrons, which can be heated by renewable electrical energy, enable the dissociation of even the most stable molecules at very modest gas temperatures.
The activation of stable molecules in LTPs is a key advantage in chemical processing but comes at a price. The selective synthesis of reaction products in these highly energetic environments remains a challenge. In the last decade, the combination of LTP with catalysts, referred to as plasma catalysis, has enabled the community to leverage the intrinsic selective nature of catalysts in the plasma environment. Several examples of critical chemical transformations, including the synthesis of ammonia and dry methane reforming, were demonstrated using this approach at near-ambient conditions.⁴
While in the above approach, near ambient temperatures are the goal, LTPs can also be engineered as efficient heat sources that enable processes at gas temperatures of more than 1000 K, ideal conditions for many thermochemical reactions. The free electrons in the plasma enable the direct Ohmic heating of the gas, which can provide major advantages over indirect heating approaches outside the reactor. For example, high-temperature conversion of CO2 and methane pyrolysis producing H2 and solid carbon have been demonstrated. Hence, plasmas can be considered as a low-emission industrial heat source, particularly for elevated temperatures that could replace conventional combustion processes and reduce associated CO2 emissions.
Next-generation manufacturing
Similar plasmas as the ones used for chemical conversion at elevated temperatures also have great potential for sustainable materials and, in particular, ore and metal processing (Fig. 2). The iron and steel-making industry is the largest industrial emitter of CO2 worldwide. Innovative iron ore reduction approaches have, to date, largely failed to replace blast furnace reduction, a major contributor to CO2 emissions in this industry. Several research teams are currently exploring and developing innovative approaches, such as plasma processes that enable a carbon-free approach to iron ore reduction.⁵ In this case, plasmas are generated in hydrogen, which is used as a reducing agent for iron oxide, producing water instead of CO2 as a reaction product.
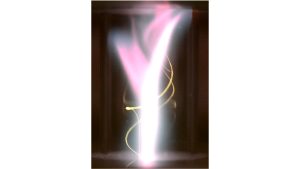
In addition to bulk commodities such as iron and steel, LTPs have great potential to synthesise nanomaterials with desired but currently uncontrollable or unattainable properties. Both plasma-enabled gas phase and liquid phase synthesis approaches are currently being explored. This includes the synthesis of metallic nanoparticles, synthetic diamonds, and silicon quantum dots (QDs). QDs have revolutionised multiple fields due to their exceptional size-dependent optical properties, as recognised by the 2023 Nobel Prize in chemistry. Extensive research has shown that plasmas can enable challenging reactions, make the process faster or less complex, and have the potential to replace current hazardous chemical processes with a clean alternative.⁶
The environment
While many of the processes described above can contribute to sustainability by reducing CO2 emissions or the use of harmful chemicals in general, also they can be leveraged for exhaust gas treatment and odour control. LTPs also enable water treatment technologies such as ozonisers and UV sources. In recent years, the pollution of water streams has significantly increased with a broad range of chemicals of concern. Many of these chemicals have established treatment processes, but a comprehensive solution remains to be implemented for per- and polyfluoroalkyl substances (PFAS), a large group of synthetic chemicals used in household and industrial products and a major global concern. PFAS are also referred to as ‘forever chemicals’ because they are not biodegradable and accumulate in the environment over time. Several chemicals of the PFAS family have been measured in concentrations sufficient to significantly impact human health and the ecological environment.
Recent studies have shown that plasmas effectively decompose PFAS, which is one of the very few technologies that seem capable of tackling this environmental challenge.⁷ The success of LTPs is attributed to electron-induced chemistry and has been shown to be highly effective for PFAS that behave as a surfactant in water and hence have elevated concentrations at the gas-liquid interface where the plasma can be generated. Pilot studies are ongoing to demonstrate the technology, while state-of-the-art research in the underpinning complex reactions and transport phenomena has the potential to significantly contribute to continued improvements in the technology.
Plasma science to drive technological advances The inherently non-equilibrium, nonlinear, and complex LTPs, producing hundreds of reactive species while interacting with substrates, have many more degrees of freedom than conventional thermochemical processes and are not holistically accessible by trial-and-error approaches. Modelling an LTP requires the description of electrical (and sometimes) magnetic fields, species transport, reaction kinetics, and energy transfer in a highly non-equilibrium framework for which electrons, ions, and neutral species can have very different energies.
In the last two decades, the LTP community has made significant advances in understanding plasma-surface interactions for semiconductor processing. Emerging applications and research areas in LTP, as described above, drastically widened the scope of plasma-material interactions to liquids and even living matter. Nonetheless, our understanding of plasma interactions with these complex ssubstrates remains limited and is significantly hindered by a lack of in-situ diagnostics and incomplete knowledge of fundamental processes occurring at these interfaces. Hence, predictive models of the most practically relevant systems are not available.
While artificial intelligence (AI) is often seen as an excellent tool to tackle complex systems and has a proven track record in the optimisation of plasma processes in the semiconductor industry, its ability in the LTP field to enable new application areas and science is yet to be established and remains unclear. The broad range of fundamental processes spanning a huge range of spatial and temporal scales, which are often highly coupled, can not only lead to highly unexpected outcomes but also new science and application opportunities.
Tackling this challenge requires convergent and interdisciplinary research that advances not only plasma science but many adjacent research areas. This will enable us to unlock new applications and educate the next generation of scientists and engineers who can translate these findings into new technologies that benefit society. The investment in fundamental research on plasma processing in the last four decades has enabled the semiconductor revolution, which is the basis of our high-tech society. This article hopefully showed that many avenues for continued success in translating fundamental research into societal impact lay ahead for the LTP field.
Acknowledgment
The author acknowledges all his collaborators, co-workers, and students who have contributed valuable and unique insights into the topics highlighted in this work.
References
National Academies of Sciences, Engineering, and Medicine. 2021. Plasma Science: Enabling Technology, Sustainability, Security, and Exploration. Washington, DC: The National Academies Press.
I. Adamovich, S. Agarwal, E. Ahedo, L.L. Alves, S. Baalrud, et al., The 2022 Plasma Roadmap: Low-Temperature Plasma Science
and Technology, Phys. D: Appl. Phys. (2022) 55 373001, https://doi. org/10.1088/1361-6463/ac5e1c
Laroussi et al, Low-Temperature Plasma for Biology, Hygiene, and Medicine: Perspective and Roadmap, IEEE Trans. Rad. Plasma Med. Sci.(2022) 6 (2) 127, https://doi.org/10.1109/TRPMS.2021.3135118.
A. Bogaerts, Plasma and plasma catalysis for sustainable chemistry. https://ieee-npss.org/wp-content/uploads/2022/02/Plasma-Connection-March-2022_FINAL.pdf
https://arpa-e.energy.gov/programs-and-initiatives/view-all-programs/rosie
P. J. Bruggeman, R. R. Frontiera, U. Kortshagen, M. J. Kushner, S. Linic, et al., Advances in plasma-driven solution electrochemistry, J. Chem. Phys. (in print)
R.K. Singh, S. Fernando, S.F. Baygi, N. Multari, S.M. Thagard, T.M. Holsen, Breakdown products from perfluorinated alkyl substances (PFAS) degradation in a plasma-based water treatment process, Environ. Sci. Technol. (2019) 53 (5) 2731–2738 https://doi.org/10.1021/acs.est.8b07031
Please note, this article will also appear in the 21st edition of our quarterly publication.
Source link