Researchers in Milan, Italy have uncovered the fundamental properties of a material of 150 million degrees by measuring essential radiation.
Nuclear fusion is the process of moving stars. It relies on the large amount of energy released when photonuclei are bound to heavier nuclei that are more closely connected. The latter shares the excess energy described by the famous Einstein relationship E = ΔMc². Here, ΔM is the difference in mass between heavy nuclei, light nuclei and C. One nuclear fusion reaction releases more than one million times more energy than traditional combustion reactions based on fossil fuels. Therefore, it is not surprising that this is the fundamental process that drives the universe.
On Earth, the most promising route to achieving nuclear fusion for energy production is to limit the fully ionized gas, called plasma, to a sophisticated magnetic cage named Tokamak. One condition is that the plasma core reaches a temperature of approximately 150 million degrees. This is about 10 times the temperature of the solar core. The other is to ensure that the plasma is high enough density to ensure that sufficient fuel is fusion. The last one is that the energy released by such a process maintains sufficient length for the system. This is to ensure that the conditions required for fusion combustion are maintained by the system itself with minimal external energy input.
Therefore, measuring temperature, more generally, more generally, the core properties of a fusion reactor is the fundamental task of the deployment of nuclear fusion as an energy source on Earth. But how do you create a measurement of an object that is expected to be at a temperature of 150 million degrees? This means that solid probes cannot be used as a thermometer, as this is most likely to be destroyed by the plasma itself.
The key to this task is to recognize that fusion plasma is a very strong source of electromagnetic and nuclear radiation. This is the energy vector of the process, including neutrons born from the fusion reaction itself, or from gamma rays spontaneously generated by several other nuclear reactions that arise primarily from the core or deceleration. . Fast electronic down in some normal scenarios.
The Neutron and Gamma Ray Diagnostic Group at Bicocca University, and the Milan, Italy-based Plasma Science and Technology Institute are global experts in the development of instruments for the measurement of neutron and gamma radiation. Confined fusion plasmas and their applications to unravel the secrets of the core of thermonuclear fusion reactors.
Measurement of neutron emission from a plasma core.
The first generation thermonuclear fusion reactor uses two isotopes of hydrogen and tritium as fuel. The fusion process between one nucleus of deuterium and one nucleus of tritium mainly releases neutrons from the core. It has energy dependent on the properties of the reacting nucleus, such as temperature and relative abundance.
In other words, similar to the spectrum of light emitted by distant stars, the energy spectrum of the neutrons of fusion origin is a fingerprint of the properties of the plasma fuel ions that determine the fusion. However, neutron measurements are not a trivial task.
Because it is not charged, neutrons are only occasionally interacted with matter, making them difficult to catch. Furthermore, when they do so, they may release only a small portion of their energy into the detector, complicating the analysis.
A key task towards the goal of measuring neutrons released by thermonuclear fusion is to ensure that appropriate spectrometers are designed and built with fine details that often rely on a particular application. For these reasons, neutron instruments can appear quite different in themselves. Some applications allow for the deployment of small detectors that can be easily integrated into Tokamac’s complex engineering environment. These can be inorganic scintillators or semiconductors, such as single crystal diamond detectors, with similar technology to those recently developed in the jewelry industry.
When broadband applications or particularly high sensitivity are required to small changes in fuel properties, more complex equipment must be designed and built, which is more required for integration into fusion reactors. Examples are flight⁸ or magnetic proton recoil equipment time, as shown in Figure 1 of the Eastern and Jet Tokamak, respectively.
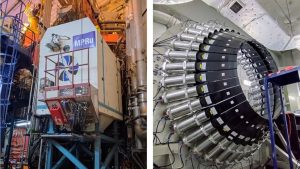
Gamma rays and energy particles
Most of the ions in the thermonuclear fusion reactor are in equilibrium at a temperature of about 150 million degrees, but a small portion of the particles has much higher energy than that. These are either fast ions produced by the fusion reaction itself or are introduced by auxiliary heating systems necessary to externally control the fusion combustion. In some normal scenarios, energy electrons (called runaway electrons) are also produced, and if not detected and mitigated, the plasma can severely damage the walls of the vacuum chambers contained in the fusion device.
All these energetic minorities need to be diagnosed, but are more difficult to detect compared to the majority of fuel ions. In a way, this is like searching for needles in a haystack, with the added complexity of the haystack being 150 million degrees!
Fortunately, the energy particles in the fusion device also emit radiation, and in most cases they emit high-energy electromagnetic radiation. These are known as gamma rays, and are either more due to other nuclear reactions than the major nuclear fusion that occurs in plasma, or so-called Bremusstral radiation, which is primarily released by runaway electrons.
Depending on the particle type and properties, the energy and intensity of gamma rays produced by the plasma will vary. The goal of the measurement is, in this case, to separate and identify the various gamma-ray energy groups that make up the overall emission, and to infer the properties of the energy particles that are responsible for production through detailed analysis. . With regard to neutrons, gamma rays sometimes interact with matter, so in many cases only a small fraction of their complete energy is released to the detector. This provides additional complications to the analysis, in addition to the inherent complexity of release due to several processes rather than one type of fusion reaction, such as neutrons.
On the other hand, gamma ray detection requires a relatively simple instrument than a neutron spectrometer. For example, a medium-sized inorganic scintillator (see Figure 2) is required. However, the design must be customized according to the measurement conditions of each device.
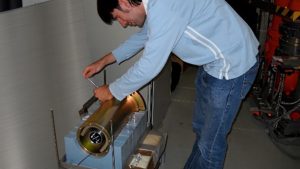
Nuclear diagnosis in the age of combustion plasma
The next step towards the ultimate goal of nuclear fusion energy production in tokamak is to generate combustion plasma. This is a special condition in which fusion combustion is maintained primarily by the heat released by the fusion reaction, if necessary in the fusion reactor, rather than in the external heating system.
Currently, several machines are being built to achieve and research such a system. The most important projects are probably the Iter in Europe, the SPARC in the US and the best in China.
In the combustion regime, plasmas become even more intense radiation sources, suggesting that neutrons and gamma ray diagnostics play a major role in elucidating complex, nonlinear phenomena that determine the dynamics of essentially self-assembled combustion plasmas. I’m doing it. of a fusion reactor.
The Milan Neutron and Gamma Ray Diagnostic Group is at the forefront of research for this fascinating new administration through the design and development of neutron and gamma Ray Independence Devices, the most important combustion plasma devices under construction.
Furthermore, younger generations of scientists have been trained at the PhD and postdoctoral level, pioneering the use of such nuclear instruments in unknown territory of burning plasma, and perhaps, perhaps, fundamentally The behavior of nuclear reactor-related plasmas for the future of energy production on Earth to contribute to the discovery of laws.
reference
Erickson, G. , &al. (2001). JET neutron emission spectroscopy – Reactions from a magnetic proton recoil spectrometer. Rev. Sci. musical instrument,,
72, 759–766. Erickson, J. , &al. (2019). Fast ions measurements of fusion plasma using neutron diagnostics at JET. Plasma Phys. control. Fusion, 61014027. Nocente, M. , &al. (2013). High-resolution gamma ray spectroscopy at MHZ count rate using LABR3 scintillator for fusion plasma applications. IEEE Transformer. Nucl. Sci. , 1408 – 1415. Nocente, M. , &al. (2020). MEV range particle physics studies of Tokamak plasma using Gamma-ray spectroscopy. Plasma Phys. control. Fusion, 62014015. Nocente, M. , &al. (2024). Space Flight: A compact spectrometer for measuring neutrons with an ASDEX upgrade tokamac. Rev. Sci. Instrum. , 95, 083501. Rigamonti, D. , &al. (2024). Jet Tokamak’s single crystal diamond-based diagnostic suite for 14 MEV neutron counts and spectroscopic measurements in DT plasma. Nucl. Fusion, 64016016. Tischler, K. (2024). Fusion of baseload energy as a future: powering a decarbonized world. Retrieved from the Innovation News Network:
https://www.innovationnewsnetwork.com/fusion-as-as-future-the-baseload-energy-powering-a-decarbonised-world/52112/ Zhang, X. , &al. (2014). Diagnosis of NB plasma in the yeast tokamac using a new time-of-flight neutron spectrometer. Nucl. Fusion, 54 104008
This article will also be featured in the 21st edition of Quarterly Publication.
Source link