The SLAC National Accelerator Laboratory leads groundbreaking advances in fusion research through innovative technology, aiming to tackle the challenges of using fusion as a viable energy source.
Since we discovered that our Sun produces energy by fusing heat atoms and releasing heat, the prospect of replicating the same process on Earth to make full use of our homes and industries is It captured the hearts of scientists.
After decades of fusion research dating back to the 1950s, the breakthrough only occurred in December 2022. Laser experiments at the National Ignition Facility (NIF) became the first laboratory fusion event to release more energy used to start it. This milestone, along with other promising approaches, drives major private investments in fusion energy, but many challenges remain before fusion expands to viable power sources.
The High Energy Density Science (HEDS) division of SLAC National Accelerator Laboratory is at the forefront of addressing these obstacles. Leveraging the unique capabilities of SLAC, the HEDS division is working with partners from academic and national research institutes to promote Inertial Fusion Energy (IFE) as a potential global energy solution.
SLAC’s LCL and MEC’s innovative contributions
The SLAC National Accelerator Laboratory has a long history of pioneering scientific advancements. Founded in 1962 to house Stanford Linear Accelerators and at the time the largest civil construction project sponsored by the US, the research at SLAC contributed to three Nobel Prizes in particle physics.
Over time, high-energy accelerators such as CERN’s large hadron colliders led the particle collision experiments where SLAC pivoted into new applications. One of its most transformative initiatives came in the early 2000s when some of the accelerators were reused in the world’s first X-ray-free electron laser, Linac Coherent Light Source (LCL).
LCLS revolutionized X-ray science by producing beams brighter than any other X-ray source. These ultra-fast, high-intensity pulses allowed researchers to capture ultra-fast processes of materials in real time. Since then, scientists around the world have used LCL to solve natural science problems, including photosynthesis, the development of next-generation painkillers, and the structure determination of viral proteins. The success of LCLS has influenced the construction of similar X-ray-free electron lasers in several countries, telling a new era of scientific research.
LCLS, along with high-power optical lasers available for extreme condition (MEC) equipment problems, has also begun a new era of precision measurements in plasma and fusion science, allowing researchers to simplify the ultra-fast changes in materials under extreme conditions. I made it possible to investigate.
In MEC, optical lasers create pressure comparable to the center of the Earth and higher than the solar coronavirus. On the other hand, X-ray beams permeate these extreme states, providing a window into dynamic evolution and testing their ability to predict the internal conditions of planets, stars, and laboratory fusion plasmas.
Promoting X-ray diagnosis for fusion science
An important aspect of nuclear fusion research is the ability to directly investigate materials as high pressure and high temperature conditions are reached. X-ray diagnostics provide essential insight into these conditions.
X-ray diffraction: By capturing atomic-scale structural changes, X-ray diffraction allows us to see how atoms in the material are rearranged under extreme compression. For example, experiments using laser-driven plastic samples revealed how the hydrocarbon chains are divided, and have been reassembled into diamonds under conditions similar to those of Uranus and Neptune. These findings can help improve planetary layer models and may also motivate new technological applications on Earth, such as the use of laser-formed nanodiamonds for biomedical and industrial purposes. X-ray scattering: This technique provides a more detailed view of interactions within high temperature conditions. By measuring how X-rays acquire or lose energy when scattered, researchers can directly determine the sample temperature. This provides insights that previously relied on indirect modeling. Recent research using this method has improved understanding of how planetary magnetic fields are generated, how materials react to pressure and radiation, and how they are used in fusion and space exploration. We have revealed how we can improve this. X-ray Imaging: The nanometer spatial scale resolution of LCLS allows for ultra-fast imaging of laser-driven samples. Researchers in our department observed the lattice mechanics of compressed silicon and how instability forms when electrons pass through hot plasma. More recent advances allow for simultaneous imaging and diffraction, providing a more complete image of material transformation.
The Future of SLAC’s Fusion Science
With the advent of LCLS-II, SLAC is poised to push further the boundaries of fusion research. This major upgrade includes a superconducting accelerator that can provide X-ray pulses per millions of seconds. It introduces unique pulse train functionality and enables sequential X-ray snapshots with varying time separations, capturing rapid sample evolution in a single shot. The upgrade also doubles the maximum X-ray energy and penetrates the sample with heavier atoms and higher density. These advances bring researchers closer to tracking the behavior of fusion fuel capsules in unprecedented detail and understanding the conditions required for sustained fusion reactions.
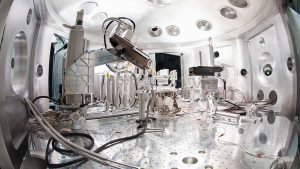
One of the key challenges of the sophistication rate fusion experiment is to provide targets at a pace that matches the laser shot rate. Previous experiments with LCLS used static arrays of hundreds of targets, but new approaches include continuous streams of liquid jets, suspended nanoparticles, or cryogenic hydrogen. , allowing experiments at much higher speeds and learning from a vast data set. These innovations support the development of next-generation fusion targets and plasma diagnostics, ultimately increasing the efficiency and survival of laser-driven radiation sources.
All of our diagnosis helps us explore the physics of fusion capsules. By the end of the decade, we are planning an upgraded laser system with issues at end stations in extreme conditions, allowing us to drive samples on the brink of fusion. The samples are driven by KJ scale laser pulses, allowing the samples to be tracked from the initial solid state to the high energy density physical regime that characterizes the inertial fusion plasma state. Our diagnosis must be collaborative in understanding optimization pathways with imaging in which X-ray diffraction investigates fuel density, scatters in search of temperature growth, and seeks instability and heterogeneity.
Sequential X-ray pulses apply these features to study how material passes through high energy density topological spaces, even when the initial target has unique properties such as defects, inclusions, and textures. I will. Machine learning combines data from these diagnostics to narrow down the conditions to reach on the timescale of the shot, revealing the underlying physical mechanisms that determine the coupling of high-intensity laser beams and material.
Although LCLS offers a unique opportunity for X-ray probing, our department understands fusion targets using beams across the electromagnetic spectrum. Optical probes can use interferometers to see how quickly the laser-driven shock wave travels through the fusion fuel, but the intensity of the emission indicates the temperature at which the compression target reaches. Terahertz radiation with wavelengths close to 1 mm allows for measurement of electrical conductivity and affects the way electric fields generate and propagate within extreme conditions. This is essential for fusion approaches that use magnetic fields to promote compression. Similar to the range of X-ray diagnostics, our experiments must combine data from all these different measurements to understand the coupling of heating and lasers to the fusion target. Beyond that, the generation of relativistic electrons, high-energy X-γ rays, magnetic fields, and energy particle beams is all probing with LCLS X-ray beams in areas of importance that are important for fusion energy science. It is an area that leads to new discoveries. development.
We are working together for a fusion-driven future
Beyond diagnostic and experimental advances, SLAC’s HEDS division works closely with theorists and computational scientists to refine models of fusion dynamics. By comparing actual experimental data with simulations, researchers can identify gaps in theoretical understanding and facilitate improvements in predictive models. Despite the fact that NIF lasers can reach the most exciting conditions, LCLS’ high-resolution data complements it and acts as an important benchmark.
The challenges of designing functional fusion power plants in the future are expanding beyond the reaction itself. The fuel – the mix of deuterium and tritium – must be reliably contained and delivered at high repetition rates. Unlike NIF, where the fused fuel is wrapped in a complete diamond shell, it is meticulously grown for several months, but commercial fusion plants require target production at 10 times faster per second. Masu.
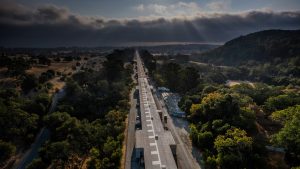
To meet this need, SLAC, in collaboration with the US Department of Energy’s Inertial Fusion Energy Network (IFE-STAR) and Fusion Innovation Research Engine Collaboratives, develops new lightweight foams that can hold cryogenic fusion fuels and It is characterized. Early LCLS experiments have already investigated the structural integrity of these foams, and future studies have shown how they retain cryogenically cooled liquid fuels, and what wet foams are under laser compression. I plan to analyze whether to act like this.
Finally, the success of the fusion force depends on materials that can withstand intense neutron bombardment produced by the deuterium-tritium fusion reaction. SLAC researchers actively collaborate with materials scientists to develop radiation-resistant alloys, and use LCL to assess changes in properties under extreme conditions, where atoms are converted by high-energy neutrons Because of that. These efforts inform the design of nuclear reactor walls that can withstand long-term exposure to fusion-driven neutron fluxes.
Conclusion
As SLAC fusion research progresses, each step approaches unleashing the power of the stars for clean, sustainable energy. With cutting-edge X-ray diagnostics, high-energy density physics experiments and deep collaboration across science, the HEDS department paves the way for a future in which fusion energy becomes a practical reality. By improving experimental methods, strengthening theoretical models, and addressing the challenges of engineering, SLAC contributes to one of the most transformative scientific efforts of the 21st century.
This article will also be featured in the 21st edition of Quarterly Publication.
Source link